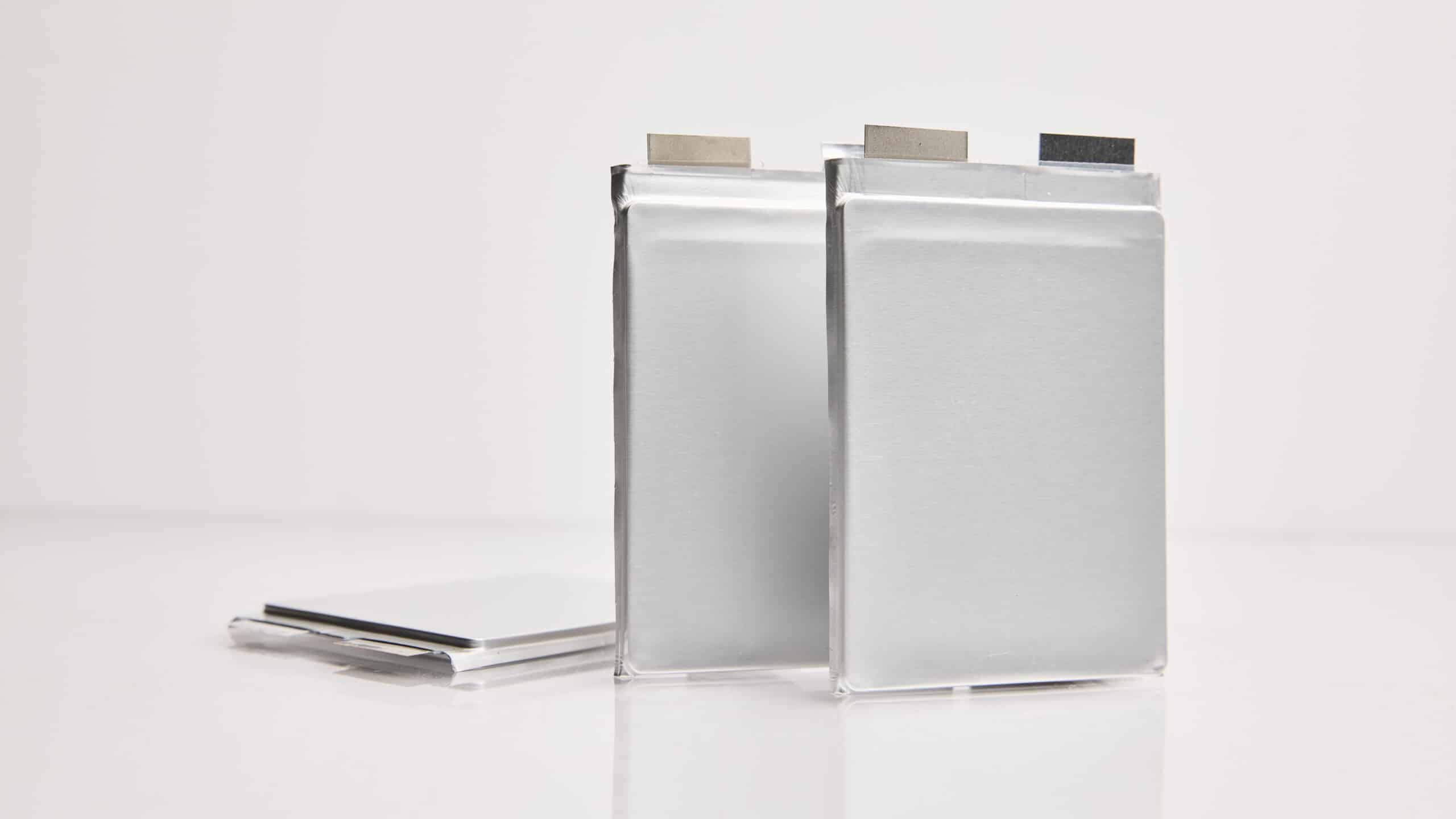
Our Strategic Blueprint
I took on the CEO role last year because I see QuantumScape as the global leader in solid state battery technologies with an ability to revolutionize energy storage and create tremendous shareholder value in the process.
Updated March 22, 2021
Following the announcement of QuantumScape’s solid-state lithium-metal battery technology results in December 2020, there has been a lot of excitement in the industry related to the potential of this new technology and the impact it could have on the automotive EV powertrain.
As with any technology, when there is a lot of excitement, there can also be a lot of misconceptions. The purpose of this paper is to help investors, customers, and others interested in this technology to understand the broader technology landscape of solid-state battery technology.
We start with a quick review of how conventional lithium-ion batteries work. As shown in the figure below, a lithium-ion battery consists of three main layers: A cathode, or positive electrode, consisting of a lithium containing mixed-metal oxide material; an anode, or negative electrode, consisting of carbon or a mix of carbon and silicon; a separator, an electrical insulator made of a porous polymer material; and an electrolyte, the medium through which lithium ions move through the battery, typically consisting of a hydrocarbon solvent and dissolved lithium salt. (In this document, we will use “carbon anode” to mean either a pure carbon anode or a hybrid carbon-silicon anode.)
A battery may be envisioned as the electrochemical equivalent of rolling a ball uphill, which requires work to be put into the system, increasing potential energy in the system during the process, and letting it roll back downhill on its own, to release stored energy and do useful work. In a fully-discharged cell, the lithium in the cell resides in the cathode, the “downhill” state. When the cell is charged, work is put into the system to drive lithium ions from the cathode to the anode, where they diffuse into the carbon particles that make up the anode. In the fully charged state the lithium ions sit in the anode, like balls that have been rolled uphill, waiting until they can be freed to roll back downhill again. When the battery is discharged, these lithium ions are allowed to move back from the anode to the cathode, and in the process, energy can be extracted from the system, just like the ball rolling downhill can release its stored energy in the form of useful work.
A next generation battery may include next generation cathode material or next generation anode material (or both). The chart below, a paper published by a group from BMW, shows a dozen different next generation cathode materials and three different anode materials. What this chart makes clear is that the energy density gains from using next-generation cathode materials are limited, unless lithium metal is used as the anode. The principal reason for this limitation is that higher capacity cathodes need correspondingly thicker anodes to hold the increased amount of lithium, drowning out some of the benefits of the cathode improvement.
Traditional lithium-ion cells use a hosted anode in which the host material, such as carbon or silicon, provides a structure to hold the lithium. For example, in the case of carbon, it takes six carbon atoms to hold one lithium atom. If, however, an anode of pure metallic lithium were to be used, as shown in the schematic below, all the carbon in the anode could be eliminated and the energy could be stored in a much smaller volume, thereby dramatically increasing the energy density of the cell.
The potential increase in energy density for a lithium-metal anode battery has been known since the mid-1970s. However, it has also been known that lithium-metal anodes do not work with conventional liquid electrolytes due to the twin issues of dendrite formation when a battery is being charged and rapid impedance growth from a chemical side-reaction between the liquid electrolyte and the lithium metal. Dendrites are needle-like formations of lithium metal which can grow across the separator and short-circuit the cell. Impedance refers to the internal resistance of the cell; growth in this resistance reduces the energy capacity of the cell as well as its ability to work at high rates of power.
Thus, it is widely believed that to make a lithium-metal anode battery, one needs a solid-state separator which is roughly as conductive as a liquid but resists dendrite formation and does not react with metallic lithium. For 40+ years, the industry has been searching for such a material.
It turns out that the lithium-metal anode enabled by such a solid-state separator could address not only the energy density problem, but also a number of the other limitations of conventional lithium-ion batteries, since many of these stem from the carbon anode as well, including:
This is the promise of solid-state lithium-metal batteries and is why the industry is so excited about the possibilities. However, making the solid-state ceramic separator required to deliver on this promise has turned out to be a very difficult challenge. More specifically, such a separator needs to:
(a) have lithium-ion conductivity similar to or better than today’s liquid electrolytes;
(b) be chemically and electrochemically stable to lithium metal; and
(c) resist the formation of lithium-metal dendrites.
Despite decades of work, the industry has not found any separator materials capable of meeting these requirements.
Many classes of separator materials have been tried, none of which have been shown to simultaneously meet the key requirements. These include:
It is important to note that if one has a system using any of the foregoing separator materials, it is still possible to make cells and report cycling results, but this cycling has to be done under compromised test conditions. In particular, the following are some of the most used compromises:
Recently, there have been a number of announcements and claims relating to solid-state batteries. The first question to ask when evaluating solid-state cell claims, is whether the cells use a lithium-metal anode or a conventional hosted (carbon or carbon-silicon) anode. If they do use a hosted anode, the key performance metrics for these batteries will be similar to conventional lithium-ion batteries and not realize the benefits of the solid-state lithium-metal approach (significantly higher energy density, fast charge, life, and cost). A number of recent claims fall into this category.
If the solid-state cell in question does use a lithium-metal anode, the next question to ask is whether it can perform under uncompromised test conditions, including near and below room temperature and high current density (i.e., high rates of power such as 1-hour charge or 15-minute charge). Specifically, what cycle life does the cell deliver at near-room temperature (~ 30°C) with automotive rates of power (>3 mA/cm2, required for one hour charge)? If the cells cannot perform under these conditions, we believe they are not commercially viable. Many of the other solid-state lithium-metal announcements fall into this category.
Many solid-state announcements either do not show any data at all, or leave out some of the above parameters when they report data, leaving an incomplete picture at best. At QuantumScape, we have developed a solid-state ceramic separator capable of meeting these requirements without requiring the compromised test conditions described above. We have presented data showing single-layer versions of our solid-state lithium-metal cells can cycle more than 1000 cycles and retain over 90% of their initial energy when cycling at aggressive 1C rates of power, near room temperature, and with modest pressure. More recently, we have presented data showing multilayer cells cycling to close to 800 cycles with similar capacity retention.
We have compiled data on key performance metrics across major solid-state battery technology efforts based on information we have been able to obtain, infer or derive from publicly disclosed materials and presentations. This data is presented in the chart below, and is current as of March 4, 2021.
We hope that this paper helps our stakeholders understand the broader technology landscape of solid-state battery technology and QuantumScape’s distinctive approach.
Forward-Looking Statements
This article contains forward-looking statements within the meaning of the federal securities laws and information based on management’s current expectations as of the date of this current report. All statements other than statements of historical fact contained in this article, including statements regarding the future development of QuantumScape’s battery technology, the anticipated benefits of QuantumScape’s technologies and the performance of its batteries, and plans and objectives for future operations, are forward-looking statements. When used in this current report, the words “may,” “will,” “estimate,” “pro forma,” “expect,” “plan,” “believe,” “potential,” “predict,” “target,” “should,” “would,” “could,” “continue,” “believe,” “project,” “intend,” “anticipates” the negative of such terms and other similar expressions are intended to identify forward-looking statements, although not all forward-looking statements contain such identifying words.
These forward-looking statements are based on management’s current expectations, assumptions, hopes, beliefs, intentions, and strategies regarding future events and are based on currently available information as to the outcome and timing of future events. These forward-looking statements involve significant risks and uncertainties that could cause the actual results to differ materially from the expected results. Many of these factors are outside QuantumScape’s control and are difficult to predict. QuantumScape cautions readers not to place undue reliance upon any forward-looking statements, which speak only as of the date made. Except as otherwise required by applicable law, QuantumScape disclaims any duty to update any forward-looking statements. Should underlying assumptions prove incorrect, actual results and projections could differ materially from those expressed in any forward-looking statements. Additional information concerning these and other factors that could materially affect QuantumScape’s actual results can be found in QuantumScape’s periodic filings with the SEC. QuantumScape’s SEC filings are available publicly on the SEC’s website at www.sec.gov.
I took on the CEO role last year because I see QuantumScape as the global leader in solid state battery technologies with an ability to revolutionize energy storage and create tremendous shareholder value in the process.
QuantumScape’s planned first commercial product, QSE-5, is a ~5 amp-hour cell designed to meet the requirements of automotive applications. Here, we’ll walk through the various elements of the cell specifications and explain some of the complexities behind the seemingly simple metric of energy density.
I took on the CEO role last year because I see QuantumScape as the global leader in solid state battery technologies with an ability to revolutionize energy storage and create tremendous shareholder value in the process.
QuantumScape’s planned first commercial product, QSE-5, is a ~5 amp-hour cell designed to meet the requirements of automotive applications. Here, we’ll walk through the various elements of the cell specifications and explain some of the complexities behind the seemingly simple metric of energy density.
Privacy Policy | Terms of Use
© 2025 QuantumScape Battery, Inc.
1730 Technology Drive, San Jose, CA 95110
info@quantumscape.com
Pamela Fong is QuantumScape’s Chief of Human Resources Operations, leading people strategy and operations, including talent acquisition, organizational development and employee engagement. Prior to joining the company, Ms. Fong served as the Vice President of Global Human Resources at PDF Solutions (NASDAQ: PDFS), a semiconductor SAAS company. Before that, she served in several HR leadership roles at Foxconn Interconnect Technology, Inc., a multinational electronics manufacturer, and NUMMI, an automotive manufacturing joint venture between Toyota and General Motors. Ms. Fong holds a B.S. in Business Administration from U.C. Berkeley and a M.S. in Management from Stanford Graduate School of Business.